Creating an account has many benefits:
- See order and shipping status
- Track order history
- Check out faster
RNA Viruses Triggered Signal Pathway
RNA Viruses Triggered Signal Pathway
Figure: RIG-I recognizes RNA viruses by detecting short dsRNAs with 5 'triphosphate terminus. MDA5 recognizes RNA viruses by detecting long dsRNAs. LGP2 positively regulates RIG-I and MDA5-mediated virus recognition. Ubiquitin ligase TRIM25 positively regulates RIG-I activation and RNF125 negatively regulates RIG-I activation. RIG-I and MDA5 interact with IPS-1 through the CARD domain. IPS-1 activates downstream signaling proteins TRAF3, TBK1/IKK-i and IRF3/IRF7 to induce type I ifn. Meanwhile, the IPS-1 signaling pathway induces NF-κB nuclear translocation through TRADD, FADD and caspase-8/-10. The cleaved fragment of caspase-8/-10 activates NF-κB.
Background
Viruses infect cells and activate innate immune signals. Innate immunity induces the production of type I interferon and an inflammatory response to clear the virus from the cell. Viruses are divided into DNA viruses and RNA viruses. DNA viruses are recognized by TLRs (toll-like receptors) and cGAS(cyclic GMP-AMP synthetase). RNA viruses, on the other hand, are recognized by RLRs (RIG-I like receptors), such as RIG-I, MDA5, and LGP2. Both TLRs and RLRs belong to pattern recognition receptors (PRRs). Innate immune signals triggered by these PRRs lead to transcriptional expression of inflammatory mediators that coordinate the elimination of pathogens and infected cells.
The nucleic acid of RNA viruses is single stranded RNA (ssRNA) or double stranded RNA (dsRNA). Many human diseases are caused by RNA viruses, including influenza, the common cold, hepatitis C, SARS, Ebola hemorrhagic fever, West Nile fever, polio, and more. It is important for the body to kill viruses and prevent diseases by antiviral reaction. Innate immunity is the most critical process in antiviral response.
RIG-I, MDA5(melanoma differentiation related gene 5), and LGP2 belong to the RLR (RIG-I like receptor) family. RLR family proteins consist of a C-terminal regulatory domain, a central DEAD box helicase/ATPase domain, and two cards (N-terminal caspase recruitment domains). They recognize genomic Rnas, such as those produced by dsRNA viruses or by replication intermediates of ssRNA viruses. RLRs are localized in the cytoplasm. Viral infection or type I interferon stimulation significantly enhanced the expression of RLRs.
RIG-I deficient cells, such as cdc and mouse fibroblasts, do not produce type I ifn and inflammatory cytokines effectively in response to many RNA viruses. These RNA viruses include Sendai virus (SeV), vesicular stomatitis virus (VSV), Japanese encephalitis virus (JEV), Newcastle disease virus (NDV), influenza virus, etc.
In contrast, MDA5-deficient cells responded normally to the above RNA viruses. However, MDA5-deficient cells lack IFN response to some picornavirridae families, such as Mongo virus, Taylor virus, and cerebral myocarditis virus (EMCV), while RIG-I-/- cells do not.
Neither RIG-I nor MDA5 is required for identification of Dengue virus and West Nile virus, whereas RIG-I is required for identification of Hepatitis C virus (HCV). Reovirus is a double-stranded segmented RNA virus that promotes IFN production primarily through MDA5. However, the absence of either RIG-I or MDA5 completely reduced IFN production, suggesting that both RIG-I and MDA5 are essential for the recognition of reovirus. RNA virus infection with RIG-I-/ -MDA5 -/ -MEF (mouse embryonic fibroblasts) does not produce type I ifn. This means that both RIG-I and MDA5 are necessary and sufficient to induce type I IFN production in response to RNA viruses.
Identification of RNA virus signaling pathways
Short Dsrnas (up to 1 kb) are recognized by RIG-I, and type I ifn induction activity is greatly enhanced by the presence of its 5 'triphosphate. We assume that RIG-I ligand is a 5 'triphosphate ssRNA produced in vitro. However, the chemically synthesized 5 'triphosphate ssRNA does not stimulate RIG-I, meaning that RIG-I activation requires double-stranded RNA. Type I IFNs can be strongly induced by dsRNA with a minimum length of 19~21mer and a terminal of 5 'triphosphate. In addition, synthetic dsRNAs with either no 5 'phosphate terminus or 5' monophosphate terminus can also activate RIG-A. This suggests that the 5 'triphosphate terminus is not always necessary to activate RIG-I, but that type I ifn production is lower than that of dsRNA with the 5' triphosphate terminus.
The presence of dsRNA is critical for RIG-I recognition. For example, VSV-infected cells can produce dsRNA, and IFN-b induction activity is reduced when the produced dsRNA is destroyed. The dsRNA fragment produced by VSV infection is about 2~2.5 kb, which is much shorter than that of VSV genomic RNA. Vsv-infected cells produce DI(defect interference) particles, which are about 2.2 kb in size. Therefore, the dsRNA fragments produced by VSV infection may be derived from DI particles. However, further research is needed to confirm this hypothesis. We know that DI granules strongly induce type I IFNs in VSV-infected cells, and RIG-I must play an important role in detecting the presence of dsRNA in DI granules. In contrast, dsRNA is difficult to detect in cells infected with influenza virus. Influenza virus genome RNA loss, after phosphatase treatment to remove interferon, is to induce interferon activity. Therefore, we speculate that RIG-I is necessary to recognize the 5 'triphosphate terminal of influenza virus.
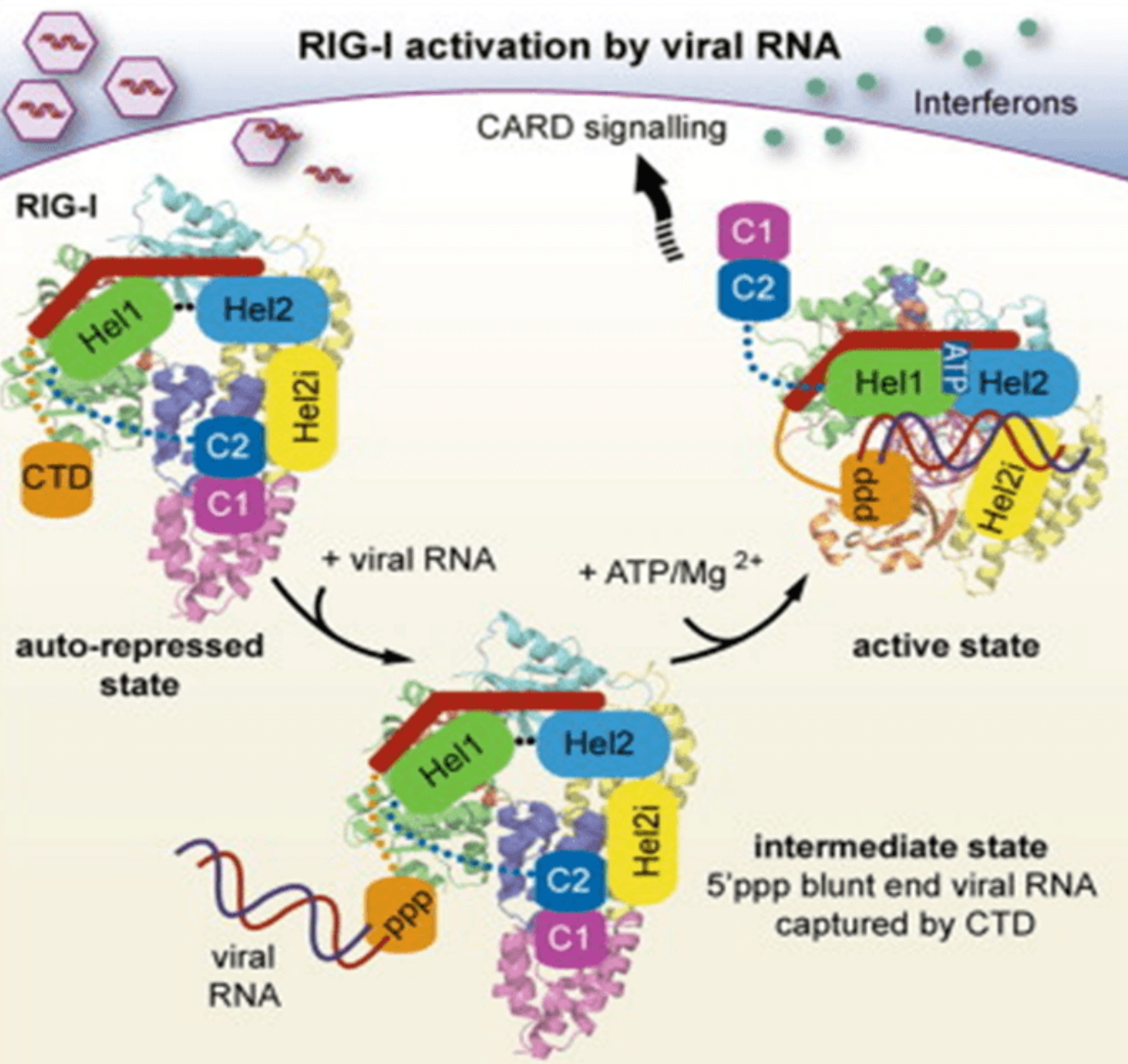
Unlike RIG-I, MDA5 detects long Dsrnas (greater than 2 kb), such as poly I:C. MDA5-/- mice showed a strong reduction of type I ifn in vivo in response to poly I:C stimulation, but not IL-12. Poly I:C severs the length of Poly I:C from the MDA5 ligand to RIG-I ligand by DsrNA-specific nuclease. This suggests that long rather than short Dsrnas can be recognized by MDA5.
Another member of the RLR family, LGP2, lacks the CARD domain. In vitro studies have shown that LGP2 is a negative regulator of RIG-I and MDA5 signaling pathways. LGP2 can separate dsRNA and inhibit RIG-I conformational change. However, in vivo studies in LGP2-/- mice have shown that LGP2 positively regulates RIG-I and MDA5-mediated type I ifn production. However, LGP2 is dispensable for type I IFN production after synthetic rna stimulation.
The C-terminal regulatory domain (RD) of the RLRs family is used for binding with dsRNAs. Recent studies have shown that RIG-I and LGP2 have large basal RDS that can form RNA-binding rings. The end of the dsRNA binds to the rna binding ring. However, MDA5 C-terminal RD is different from RIG-I and LGP2. MDA5 RD also has a large basal surface, but does not form rna binding rings. Therefore, the RNA-binding activity of MDA5 is much weaker than RIG-I and LGP2. The DExD/H helicase domain of RLRs catalyzes ATP-induced production of type I IFN.
RNA viruses trigger the downstream signaling pathway of IPS-1
Activation of RLR signals requires RIG-I conformational modification. RIG-I cosynthesis can be regulated by ubiquitination. K63-linked RIG-I polyubiquitination is mediated by E3 ubiquitin ligases RNF135 and TRIM25.
The RLRs adapter IPS-1 (ifn-b promoter stimulator 1), also known as MAVS or VISA, has an n-terminal CARD-domain. The card field interacts with the rlr card to trigger the signal axis. IPS-1 is located on the mitochondrial membrane. When it is cleaved from mitochondria by HCV NS3/4A protease, RLR signaling activity is lost. NOD9 has been reported as an inhibitor of IPS-1 and is a member of the NLR family located on mitochondria, but recent studies have shown that NOD9 is responsible for the production of reactive oxygen species. Therefore, the relationship between NOD9 and RLR signaling pathway needs to be further studied. IPS-1 activates downstream proteins TRAF3 and TRADD. TRAF3 activates IKK-i/TBK1 (TANK-binding kinase 1), which then dimers and transmits signals to downstream proteins IRF3(interferon regulatory factor 3) and IRF7(interferon regulatory factor 7), which are phosphorylated into the nucleus and induce type I interferon production. The other signal is activated by TRADD and FADD. TRADD and FADD transmit signals leading to cleavage of caspase-8/-10. This process induces cytokine production by activating NF-κB. Both the TRIF and IPS-1 signaling pathways regulate IFN production and the expression of IFN-inducing genes.
Type I ifn expression is regulated by activation of NF-κB, IRF3, and IRF7. Among these factors, IRF3 and IRF7 are activated during viral infection and mainly induce type I ifn production. In contrast, NF-κB is activated in response to stimulation, modulating the expression of inflammation, and they are also involved in inducing the production of type I IFNs, interacting with IRF3 and IRF7.
Reference
1. Takeuchi, O., and Akira, S. (2009). Innate immunity to virus infection. Immunol. Rev. 227, 75–86.
2. Yoneyama, M., and Fujita, T. (2008). Structural mechanism of RNA recognition by the RIG-I-like receptors. Immunity 29, 178–181.
3. Kato, H., Takeuchi, O., Sato, S., Yoneyama, M., Yamamoto, M., Matsui, K., Uematsu, S., Jung, A., Kawai, T., Ishii, K.J., et al. (2006). Differential roles of MDA5 and RIG-I helicases in the recognition of RNA viruses. Nature 441, 101–105.
4. Kato, H., Takeuchi, O., Mikamo-Satoh, E., Hirai, R., Kawai, T., Matsushita, K., Hiiragi, A., Dermody, T.S., Fujita, T., and Akira, S. (2008). Length-dependent recognition of double-stranded ribonucleic acids by retinoic acid-inducible gene-I and melanoma differentiation-associated gene 5. J. Exp. Med. 205, 1601–1610.
5. Sumpter, R., Jr., Loo, Y.M., Foy, E., Li, K., Yoneyama, M., Fujita, T., Lemon, S.M., and Gale, M., Jr. (2005). Regulating intracellular antiviral defense and permissiveness to hepatitis C virus RNA replication through a cellular RNA helicase, RIG-I. J. Virol. 79, 2689–2699.
6. Hornung, V., Ellegast, J., Kim, S., Brzozka, K., Jung, A., Kato, H., Poeck, H., Akira, S., Conzelmann, K.K., Schlee, M., et al. (2006). 50-Triphosphate RNA is the ligand for RIG-I. Science 314, 994–997.
7. Pichlmair, A., Schulz, O., Tan, C.P., Naslund, T.I., Liljestrom, P., Weber, F., and Reis e Sousa, C. (2006). RIG-I-mediated antiviral responses to single-stranded RNA bearing 50-phosphates. Science 314, 997–1001.
8. Takahasi, K., Yoneyama, M., Nishihori, T., Hirai, R., Kumeta, H., Narita, R., Gale, M., Jr., Inagaki, F., and Fujita, T. (2008). Nonself RNA-sensing mechanism of RIG-I helicase and activation of antiviral immune responses. Mol. Cell 29, 428–440.
9. Pattnaik, A.K., Ball, L.A., LeGrone, A., and Wertz, G.W. (1995). The termini of VSV DI particle RNAs are sufficient to signal RNA encapsidation, replication, and budding to generate infectious particles. Virology 206, 760–764.
10. Hsu, M.T., Parvin, J.D., Gupta, S., Krystal, M., and Palese, P. (1987). Genomic RNAs of influenza viruses are held in a circular conformation in virions and in infected cells by a terminal panhandle. Proc. Natl. Acad. Sci. USA 84, 8140–8144.
11. Rothenfusser, S., Goutagny, N., DiPerna, G., Gong, M., Monks, B.G., Schoenemeyer, A., Yamamoto, M., Akira, S., and Fitzgerald, K.A. (2005). The RNA helicase Lgp2 inhibits TLR-independent sensing of viral replication by retinoic acid-inducible gene-I. J. Immunol. 175, 5260–5268.
12. Satoh, S., Kato, H., Kumagai, Y., Yoneyama, M., Sato, S., Matsushita, K., Tsujimura, T., Fujita, T., Akira, S., and Takeuchi, O. (2010). LGP2 is a positive regulator of RIG-I- and MDA5-mediated antiviral responses. Proc. Natl. Acad. Sci. USA 107, 1512–1517.
13. Cui, S., Eisenacher, K., Kirchhofer, A., Brzozka, K., Lammens, A., Lammens, K., Fujita, T., Conzelmann, K.K., Krug, A., and Hopfner, K.P. (2008). The C-terminal regulatory domain is the RNA 50-triphosphate sensor of RIG-I. Mol. Cell 29, 169–179.
14. Gack, M.U., Shin, Y.C., Joo, C.H., Urano, T., Liang, C., Sun, L., Takeuchi, O., Akira, S., Chen, Z., Inoue, S., and Jung, J.U. (2007). TRIM25 RING-finger E3 ubiquitin ligase is essential for RIG-I-mediated antiviral activity. Nature 446, 916–920.
15. Kawai, T., and Akira, S. (2006). Innate immune recognition of viral infection. Nat. Immunol. 7, 131–137.
16. Arnoult, D., Soares, F., Tattoli, I., Castanier, C., Philpott, D.J., and Girardin, S.E. (2009). An N-terminal addressing sequence targets NLRX1 to the mitochondrial matrix. J. Cell Sci. 122, 3161–3168.
Aladdin:https://www.aladdinsci.com
- read more
- read more
- read more
- read more