Creating an account has many benefits:
- See order and shipping status
- Track order history
- Check out faster
Versatile Cell Culture Scaffolds via Bio-orthogonal Click Reactions
Introduction
In the field of tissue engineering and regenerative medicine, the design of biomaterial scaffolds that can reproduce the complex extracellular nature of living tissues in three dimensions is a challenging requirement. Hydrogels are a widely studied and developed class of synthetic extracellular matrix mimics (ECM). Due to their high water content, sufficient elasticity and ease of transfer, they are suitable for cell culture in 2D and 3D environments and serve as carriers for some key mechanical properties testing in simulated soft tissue environments.1-5
In general, hydrogels can be prepared from a variety of natural and synthetic precursors that can be cross-linked by physical, ionic, or covalent interactions.2 When cells are incubated in such systems, hydrogels formed from natural polymers are capable of providing them with a large number of biological signals (e.g., cell adhesion proteins, growth factor repertoire, protein degradation domains), but are generally less flexible in their structure, consisting of repetitive units that control the physical and chemical properties of the resulting material. In addition, hydrogels formed from synthetic macromolecules allow precise control of some key parameters on tissue engineering such as mechanics, swelling, and degradation, but often these chemical components are not recognized by cells. Therefore, there is a growing interest in the development of synthetic gels as cell carriers and culture systems that can effectively integrate the advantages of synthetic and natural materials. In this paper, some concepts involved are discussed based on the research progress of PEG hydrogel. Peg hydrogels have been widely used in 2D and 3D cell cultures, and various methods and theories have been used to control the properties of PEG gels and integrate cellular information.3-5 More importantly, the PEG material is able to effectively reduce non-specific protein interactions, allowing researchers to better understand how cells receive specific signals from the external cellular environment.
CHEMISTRY AND MOLECULAR DESIGN PRINCIPLES FOR PEG HYDROGEL SYNTHESIS
Usually under aqueous conditions, PEG hydrogels are formed from covalently or non-covalently crosslinked linear or branched/star polymer chains. Physically cross-linked polyethylene glycol (PEG) and polypropylene oxide block copolymers have been widely used by forming hydrogels in situ due to their low critical dissolution temperature (LCST) (≈37 °C);6 However, the mechanical strength of these hydrogels assembled by non-covalent bonds is usually weak. To this end, researchers have found a new alternative method to construct highly stable PEG hydrogels through covalent cross-linking.2 To build covalently crosslinked networks for cell culture, one must also be mindful of the cytocompatibility of the crosslinking method. In this work, two polymerization mechanisms have been widely used: (i) chain growth and (ii) step-growth.4,5 Chain polymerization of end-functionalized PEGs is robust and simple,4 but results in a complex network structure that is structurally heterogeneous with a broad distribution of kinetic chain lengths and degradation that is limited to the network crosslink points. Recently, the step-growth polymerization mechanism of PEG has emerged as a more convenient method to achieve controlled and more homogeneous cross-linked polymer networks, while providing an easy way to introduce biological signals. In general, for PEG-based stepped-growth hydrogels, bifunctional molecules are stoichiometrically used to cross-link multifunctional molecular systems; In general, the basic condition for gel formation is that its average functional degree must be greater than two. Depending on the desired grid size or cross-link density, either or both of these molecules can be derived from PEG-based polymers (Figure 1). For hydrogels targeting 3D cell culture and tissue regeneration, both being applicable to physiological conditions and efficient, non-toxic chemical cross-linking methods are critical requirements, as gels often need to be formed in the presence of cells and proteins. These complexities have led life scientists to explore various chemical reactions to form mild and specific hydrogels, but their reaction conditions still need to be maintained at the appropriate physiological pH and temperature.


Figure 2. Popularly known click reactions: A) Copper catalyzed azide-alkyne cycloaddition; B) Base catalyzed Michael addition reaction between thiol and maleimide; C) Photoinitiated thiol-ene coupling.
Following the above introduction to PEG hydrogels and click chemistry, the remainder of this article will focus on: (i) the construction of PEG hydrogels for cell culture using various click reactions, and (ii) how the orthogonalities of the click reactions can be exploited to precisely functionalize these materials through selective biological expression for directing cell function.
MICHAEL ADDITIONS
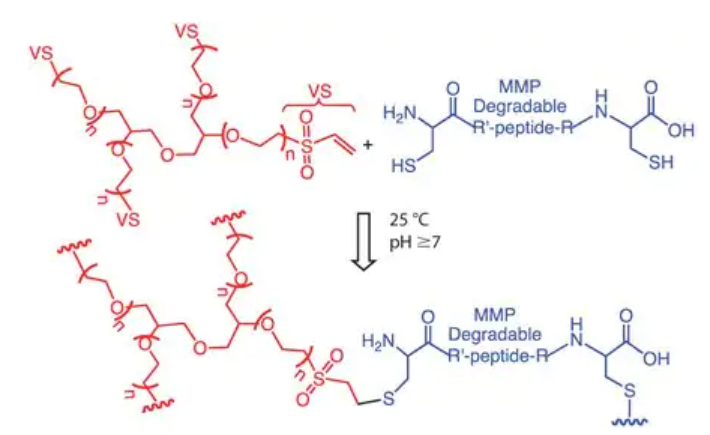
Figure 3. Step-growth hydrogel formation between 4-arm PEG tetra vinyl sulfone and cysteine end functionalized MPP cleavable peptide via Michael addition.
THIOL-ENE CHEMISTRY
Unlike Michael addition reactions (i.e., anionic), thiol-ene reactions proceed via a radical mechanism in which a thiyl radical can add over to either an electron deficient or electron rich carbon-carbon double bond (termed -ene).8 A typical thiol-ene mechanism involves (Figure 4A): (i) abstraction of a hydrogen atom from a thiol by an initiating radical, (ii) the resultant thiyl radical adding over to an alkene functionality creating a carbon radical, and (iii) chain transfer of the carbon radical to another thiol, regenerating a thiyl radical which then propagates to a new alkene. While the thiyl radicals can be generated from thermal, photo, and redox initiators, photo-initiators are especially attractive for cell relevant applications, as cytocompatible photoinitiation conditions have been widely reported8 and also allow the user to control the reaction spatially and temporally.
Another group reported the synthesis of peptide-functionalized PEG hydrogels using photoinitiated thiol-ene chemistry.13 In their approach (Figure 4), norbornene-functionalized multi-arm PEGs and cysteine containing chymotrypsin cleavable peptides were crosslinked to create PEG hydrogels, using lithium phenyl-2,4,6-trimethylbenzoylphosphinate (LAP) as a water-soluble photo initiator and 365-420 nm light in PBS buffer under physiological pH conditions. Both gel forming conditions and the resultant hydrogel system supported high levels of cell viability (>95%). More importantly, because this chemistry is based on light, photopatterning of cell signals, such as the cell-adhesive RGDS, at specific locations of the gel was achieved (Figure 4), which ultimately dictated local cell interactions and morphology. This facile incorporation of any thiolated cell signaling moieties (e.g., thiolated proteins) on hydrogel scaffolds post cell encapsulation enables experimenters the possibility of understanding and directing cellular functions (e.g., differentiation) in a spatio-temporal fashion. In a similar line of inquiry, thiol-acrylate polymerizations have also been employed to incorporate thiolated affinity peptides that retain certain cell-survival proteins in the scaffold14, as well as proteins that mimic cell-cell interactions,15 thereby, integrating additional functional properties of the ECM.
Figure 4.Thiol-ene photochemistry: A) General mechanism of the thiol-ene reaction; B) Structure of a commonly used ene, 4-arm PEG tetra norbornene; C) A cysteine containing chymotrypsin cleavable peptide crosslinker; D) Schematic of photopatterning networks formed via thiol-ene polymerization by reacting a network off-stoichiometry and subsequently conjugating a thiolated cell adhesive ligand at specific locations within the hydrogel scaffold; Image obtained from photopatterning of Alexafluor488 labeled RGD, scale bar: 500 μm.
STRAIN-PROMOTED AZIDE-ALKYNE
CYCLOADDITION REACTIONS
Among various click reactions, copper (I) catalyzed azide-alkyne cycloaddition (CuAAC) to form 1,2,3-triazole (Figure 2A) has emerged as a widely utilized reaction for applications ranging from material to biomedical sciences, because azides, alkynes, and triazoles are largely inert to various reaction conditions and to most biological systems. While this click reaction gained popularity only after the introduction of a copper (I) catalyst into classical Huisgen cycloaddition, the toxic nature of this transition metal considerably restricted its utility in biological systems. Particularly for cell culture applications, CuAAC produced hydrogels simply remained as a prefabricated cell culture substrate rather than a cell encapsulation scaffold,16,17 while being the icebreaker for the emergence of sequential click reactions (see Sequential Click Reactions section) techniques in hydrogel scaffolds.18
To overcome this limitation of CuAAC, Bertozzi and co-workers pioneered the development of strain-promoted azide-alkyne cycloaddition (SPAAC) between cyclooctynes and azides (Figure 5) which proceeds without the need for copper catalyst, making it as an ideal bioorthogonal reaction suitable for cell encapsulation.9 Building from the concept of copper-free click chemistry, PEG hydrogels have been synthesized using 4-arm PEG tetraazide (Figure 6A) and di-cyclooctyneterminated matrix metalloproteinase (MMP) degradable peptides.19,20 Here, the gel is crosslinked using a degradable peptide to enable network degradation and remodeling, as dictated by cell-secreted MMPs. As one example, a gem-difluorocyclooctyne (DIFO)9,19 was employed for its faster kinetics due to the presence of electron withdrawing fluorines, along with the characteristic ring strain (Figure 5). Gelation occurs in less than 5 minutes under physiological conditions in the presence of cells.
Figure 5. SPAAC between an azide and a difluorocyclooctyne eliminates the need for a copper catalyst and allows material fabrication under physiological conditions.
SEQUENTIAL CLICK REACTIONS
In many biological applications, one usually wants to introduce relevant functional signal cues at specific time points as well as spatially constrained regions. These signals may include survival cues (such as integrin binding molecules), growth factors (such as affinity ligands that sequentiate cytokines), or cell-specific degradation sites (such as MMP cleavable linkers used to release signals or allow cell motility). This leads to the emergence of sequential click reactions: one click chemistry is used to form the gel, followed by another click chemistry to modify it in a space-time controlled manner. Since the Thiol-ene chemical reaction is driven by light, it has emerged as a powerful and versatile method to achieve the introduction of well-defined and highly complex structures in PEG hydrogel cell niches.
Exploiting the orthogonality between SPAAC and thiol-ene click reactions: our research group has demonstrated the formation of PEG hydrogels via SPAAC (Figure 6A) followed by the creation of spatio and temporal photopatterns using the thiol-ene click reaction, in which case the peptide crosslinker carried a pendant allyl group (Figure 6A) to allow post-gelation photopatterning.19 Cells encapsulated within this hydrogel matrix not only expressed >90% cell viability, but also responded to the patterned biochemical cues. For example, spatial control over cell morphology and adhesions were established upon patterning RGD peptide at specific locations of the gel (Figure 6A). Similarly, when the self-quenched difluorescein collagenase cleavage peptide (with enhanced fluorescence after cleavage) is irradiated with light, stronger fluorescence can be observed in areas of high collagenase activity, i.e., around the cell, thus enabling real-time observation of local cell activity.
More recently, we achieved sequential patterning and depatterning of biologically relevant cues using orthogonal photocoupling and photocleavage reactions (Figure 6B and 6C).21 Spatio and temporal control over coupling and cleavage were executed using light of different wavelengths using a thiolated RGD peptide that contained a nitrobenzyl ether linker21-23 to enable the photocleavage (Figure 6D) and subsequently allowed us to externally direct cell adhesion and detachment of human mesenchymal stem cell (hMSC) populations at specific locations within the hydrogel (Figure 6B and 6C). Such dynamic control over biochemical cues on hydrogel scaffolds enables one to not only spatially control release of specific signals and cell types for cell delivery applications in regenerative medicine, but also provide opportunities to tune stem cell differentiations by controlling the presentation of specific and multiple cues in a spatio-temporal manner.
References
1. Peppas N, Hilt J, Khademhosseini A, Langer R. 2006. Hydrogels in Biology and Medicine: From Molecular Principles to Bionanotechnology. Adv. Mater.. 18(11):1345-1360. https://doi.org/10.1002/adma.200501612
2. Lee KY, Mooney DJ. 2001. Hydrogels for Tissue Engineering. Chem. Rev.. 101(7):1869-1880. https://doi.org/10.1021/cr000108x
3. Tibbitt MW, Anseth KS. 2009. Hydrogels as extracellular matrix mimics for 3D cell culture. Biotechnol. Bioeng.. 103(4):655-663. https://doi.org/10.1002/bit.22361
4. Kloxin AM, Kloxin CJ, Bowman CN, Anseth KS. Mechanical Properties of Cellularly Responsive Hydrogels and Their Experimental Determination. Adv. Mater.. 22(31):3484-3494. https://doi.org/10.1002/adma.200904179
5. Zhu J. 2010. Bioactive modification of poly(ethylene glycol) hydrogels for tissue engineering. Biomaterials. 31(17):4639-4656. https://doi.org/10.1016/j.biomaterials.2010.02.044
6. Jeong B, Kim SW, Bae YH. 2002. Thermosensitive sol?gel reversible hydrogels. Advanced Drug Delivery Reviews. 54(1):37-51. https://doi.org/10.1016/s0169-409x(01)00242-3
7. Kolb H, Finn M, Sharpless K. 2001. Angew. Chem. Int.. 40. 2004-2021. https://onlinelibrary.wiley.com/doi/10.1002/1521-3773(20010601)40:11%3C2004::AID-ANIE2004%3E3.0.CO;2-5
8. Hoyle C, Bowman C. 2010. Thiol-Ene Click Chemistry. Angewandte Chemie International Edition. 49(9):1540-1573. https://doi.org/10.1002/anie.200903924
9. Sletten E, Bertozzi C. 2009. Bioorthogonal Chemistry: Fishing for Selectivity in a Sea of Functionality. Angew. Chem. Int. Ed.. 48(38):6974-6998. https://doi.org/10.1002/anie.200900942
10. Pratt AB, Weber FE, Schmoekel HG, Müller R, Hubbell JA. 2004. Synthetic extracellular matrices for in situ tissue engineering. Biotechnol. Bioeng.. 86(1):27-36. https://doi.org/10.1002/bit.10897
11. Lutolf MP, Hubbell JA. 2003. Synthesis and Physicochemical Characterization of End-Linked Poly(ethylene glycol)-co-peptide Hydrogels Formed by Michael-Type Addition. Biomacromolecules. 4(3):713-722. https://doi.org/10.1021/bm025744e
12. Phelps EA, Enemchukwu NO, Fiore VF, Sy JC, Murthy N, Sulchek TA, Barker TH, García AJ. 2012. Maleimide Cross-Linked Bioactive PEG Hydrogel Exhibits Improved Reaction Kinetics and Cross-Linking for Cell Encapsulation and In Situ Delivery. Adv. Mater.. 24(1):64-70. https://doi.org/10.1002/adma.201103574
13. Fairbanks BD, Schwartz MP, Halevi AE, Nuttelman CR, Bowman CN, Anseth KS. 2009. A Versatile Synthetic Extracellular Matrix Mimic via Thiol-Norbornene Photopolymerization. Adv. Mater.. 21(48):5005-5010. https://doi.org/10.1002/adma.200901808
14. Lin C, Anseth KS. 2009. Controlling Affinity Binding with Peptide-Functionalized Poly(ethylene glycol) Hydrogels. Adv. Funct. Mater.. 19(14):2325-2331. https://doi.org/10.1002/adfm.200900107
15. Lin C, Anseth KS. 2011. Cell-cell communication mimicry with poly(ethylene glycol) hydrogels for enhancing -cell function. Proceedings of the National Academy of Sciences. 108(16):6380-6385. https://doi.org/10.1073/pnas.1014026108
16. Malkoch M, Vestberg R, Gupta N, Mespouille L, Dubois P, Mason AF, Hedrick JL, Liao Q, Frank CW, Kingsbury K, et al. 2006. Synthesis of well-defined hydrogel networks using Click chemistry. Chem. Commun..(26):2774. https://doi.org/10.1039/b603438a
17. Liu SQ, Rachel Ee PL, Ke CY, Hedrick JL, Yang YY. 2009. Biodegradable poly(ethylene glycol)-peptide hydrogels with well-defined structure and properties for cell delivery. Biomaterials. 30(8):1453-1461. https://doi.org/10.1016/j.biomaterials.2008.11.023
18. Polizzotti BD, Fairbanks BD, Anseth KS. 2008. Three-Dimensional Biochemical Patterning of Click-Based Composite Hydrogels via Thiolene Photopolymerization. Biomacromolecules. 9(4):1084-1087. https://doi.org/10.1021/bm7012636
19. DeForest CA, Polizzotti BD, Anseth KS. 2009. Sequential click reactions for synthesizing and patterning three-dimensional cell microenvironments. Nature Mater. 8(8):659-664. https://doi.org/10.1038/nmat2473
20. DeForest CA, Sims EA, Anseth KS. 2010. Peptide-Functionalized Click Hydrogels with Independently Tunable Mechanics and Chemical Functionality for 3D Cell Culture. Chem. Mater.. 22(16):4783-4790. https://doi.org/10.1021/cm101391y
21. DeForest CA, Polizzotti BD, Anseth KS. 2009. Sequential click reactions for synthesizing and patterning three-dimensional cell microenvironments. Nature Mater. 8(8):659-664. https://doi.org/10.1038/nmat2473
22. Kloxin AM, Kasko AM, Salinas CN, Anseth KS. 2009. Photodegradable Hydrogels for Dynamic Tuning of Physical and Chemical Properties. Science. 324(5923):59-63. https://doi.org/10.1126/science.1169494
23. DeForest CA, Polizzotti BD, Anseth KS. 2009. Sequential click reactions for synthesizing and patterning three-dimensional cell microenvironments. Nature Mater. 8(8):659-664. https://doi.org/10.1038/nmat2473
24. Nimmo CM, Shoichet MS. 2011. Regenerative Biomaterials that “Click”: Simple, Aqueous-Based Protocols for Hydrogel Synthesis, Surface Immobilization, and 3D Patterning. Bioconjugate Chem.. 22(11):2199-2209. https://doi.org/10.1021/bc200281k
Aladdin:https://www.aladdinsci.com
- read more
- read more
- read more
- read more